The power of decay
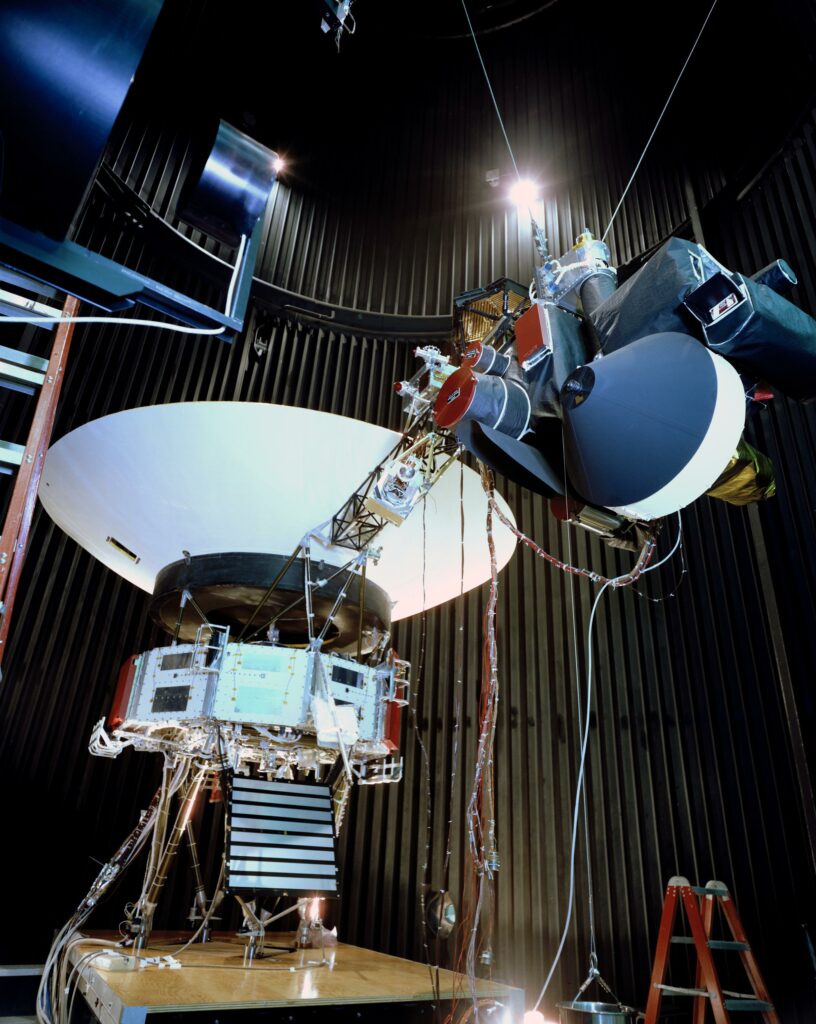
(Courtesy of NASA)
Launched on September 5, 1977, NASA’s space probe Voyager 1 is now the most distant human-made object in existence (writes Peter Donaldson). At the time of writing, the spacecraft had made it more than 14,569,656,000 miles from the Sun, a figure that continues to grow at a rate of about 38,027 mph. The probe is still transmitting data back to Earth, and four of its 10 scientific instruments are still working nearly 45 years after its launch.
The powerplant that enables such longevity consists of three radioisotope thermoelectric generators (RTGs). The RTG is without doubt the best-known of a small family of devices capable of exploiting the energy released by the decay of radioactive elements to generate electricity. Loosely speaking, they can be thought of as nuclear batteries and should therefore be useful in a few categories of EV, at least those that share Voyager’s need for modest amounts of power that can flow for years with zero maintenance.
There are different ways to convert radioactive decay into electric current. In addition to the thermoelectric principle used in RTGs, there are also thermionic and thermophotovoltaic methods and Stirling generators. Non-thermal options including electrostatic, direct charging, electromechanical, radiovoltaic (of the alpha, beta and gamma varieties), and radiophotovoltaic methods. Some are more practical than others.
Radioisotopes that have been used include tritium, nickel-63, promethium-147, technetium-99, plutonium-238, curium-242 and -244, strontium-90, and carbon-14, the latter being the energy source in what is called the nuclear diamond battery.
This is in the early stages of development by a team at the University of Bristol, in the UK. Basically it is an artificial diamond that can generate a small electric current when placed in a radioactive field, by exploiting the energy released during the beta decay of carbon-14. This carbon isotope decays to nitrogen-14 through the emission of a beta particle, which is a high-energy electron.
The diamond is in the form of a sandwich of thin layers of large polycrystalline grains laid down using chemical vapour deposition, with the C14 supplied by methane gas. The radioactive material is sandwiched between layers of non-radioactive carbon-12, which acts as shielding and prevents the radioactivity from escaping.
According to the Bristol team, current is generated when each beta particle moves into the surrounding diamond structure, creating successive electron hole pairs due to inelastic impacts with other carbon atoms and generating a cascade of less energetic electrons, which are collected at the metal contact to the diamond.
The team points out that diamond is a semiconductor whose behaviour can be compared to that of a silicon solar cell, in which current flows when valence electrons gain enough energy to move into the conduction band.
The team’s estimate is that 1 g of C14 in a diamond cell could deliver 15 J (0.0041666667 Wh) per day for the entire half-life of C14, which is about 5730 years, giving a total energy output over that time of 2.7 TJ, or 750 MWh. It’s a tiny amount of power delivered over a vast span of time but it adds up to a huge amount of energy.
Prototype nuclear diamond cells measure 10 mm2 x 5 mm thick without contacts and wiring. They are most likely to be connected into batteries with larger energy capacities and higher power outputs, and used as trickle chargers for capacitors to deliver larger amounts of power periodically or to provide continuous power for low-draw devices such as passive sensors.
As far as mobility applications are concerned, they would seem to be good candidates for tiny robots of many kinds, and long-endurance, slow-moving underwater vehicles such as buoyancy-driven gliders. The power of nuclear decay could extend the endurance of the latter from months to decades, as Voyager 1 could attest.
ONLINE PARTNERS
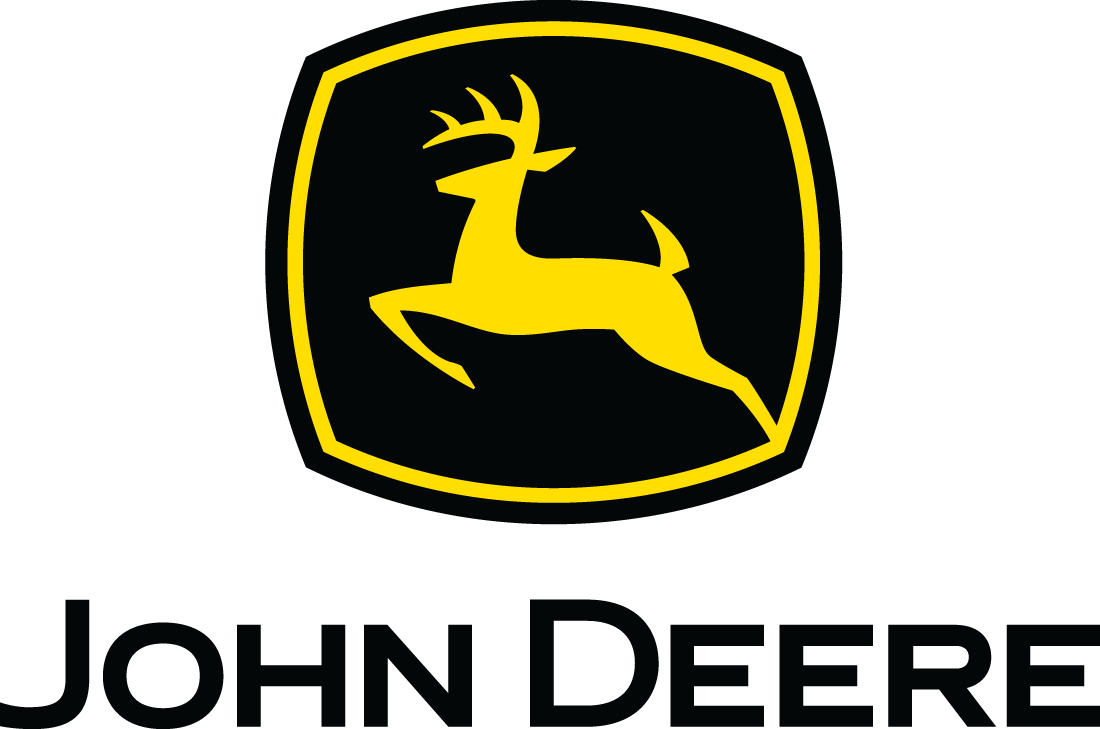
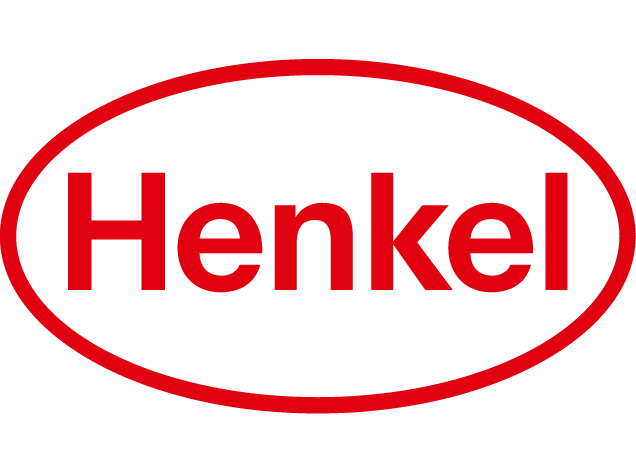
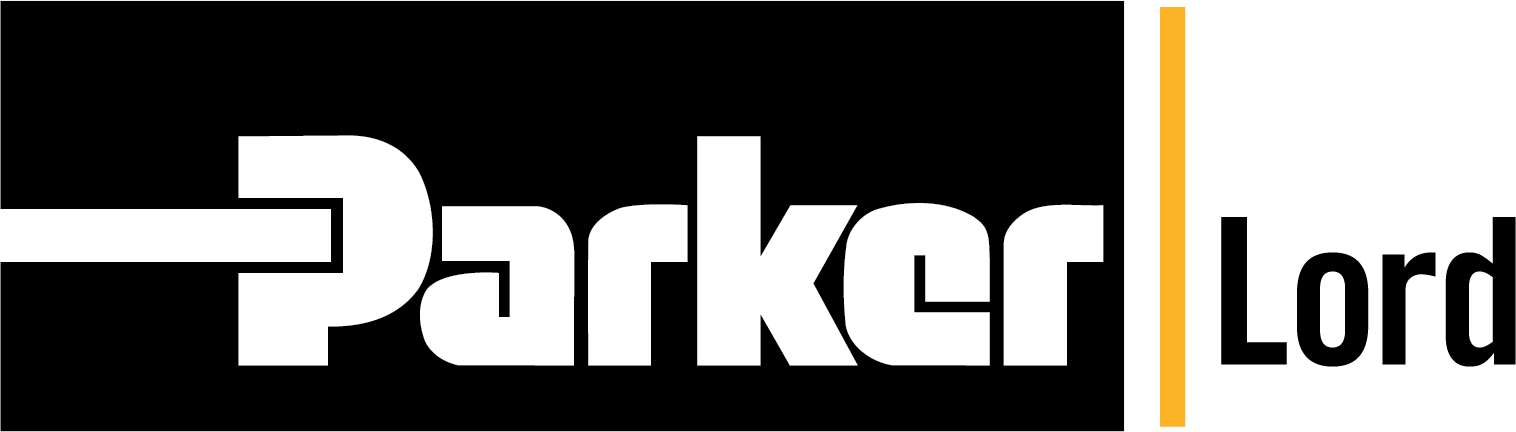
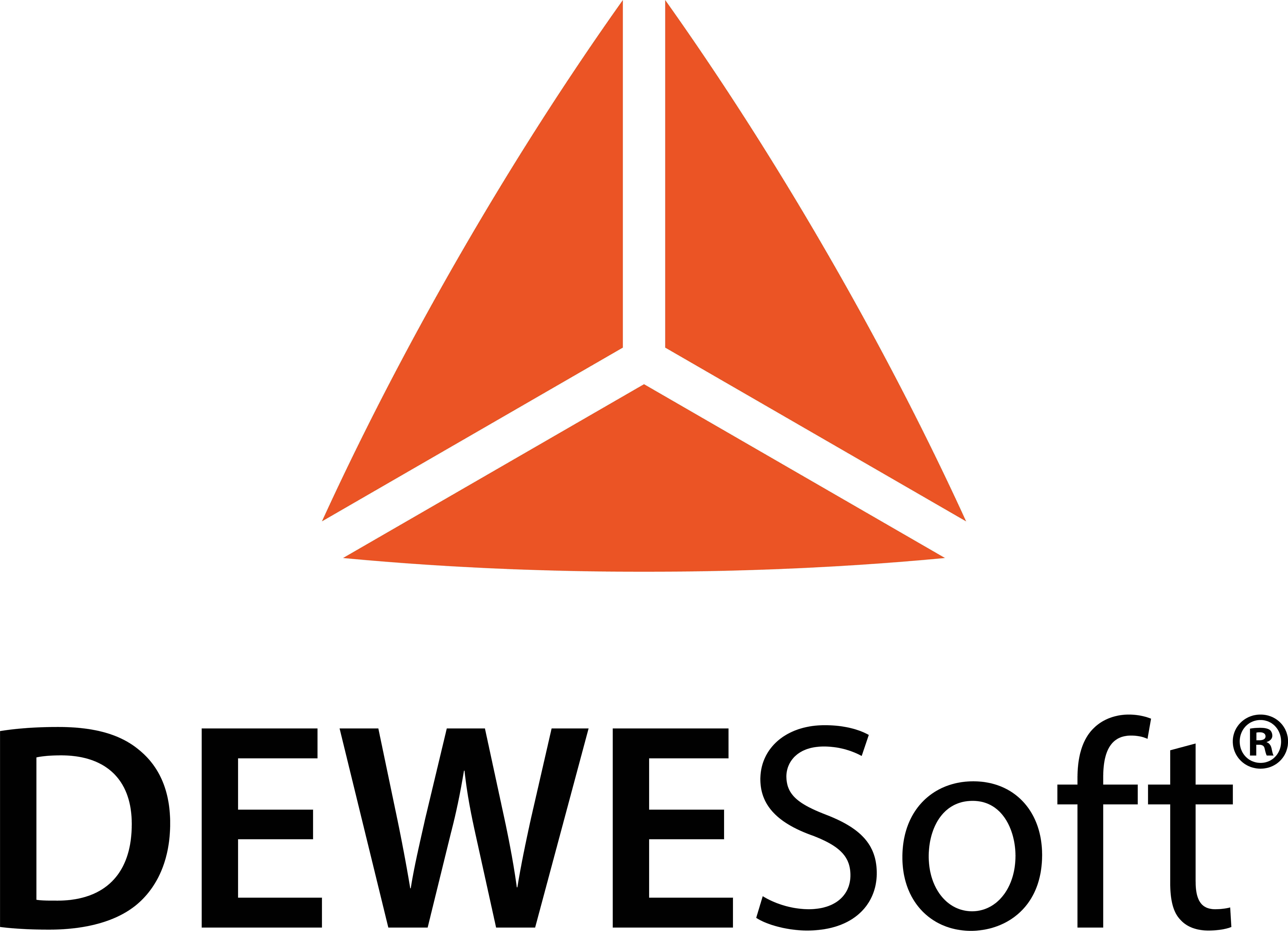
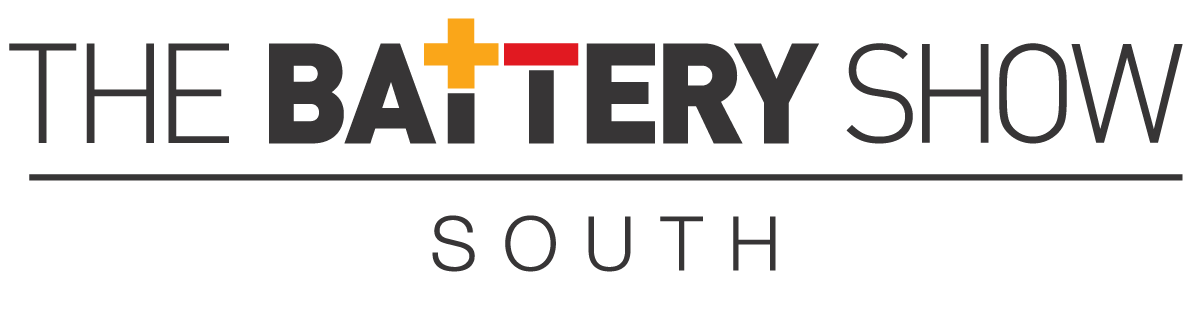
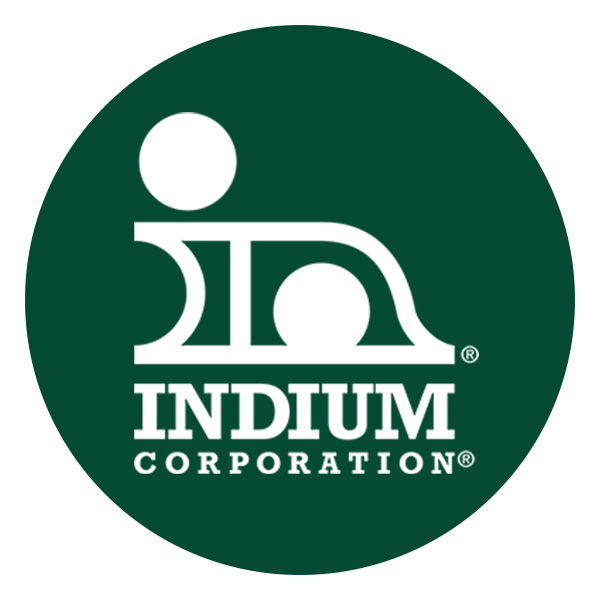

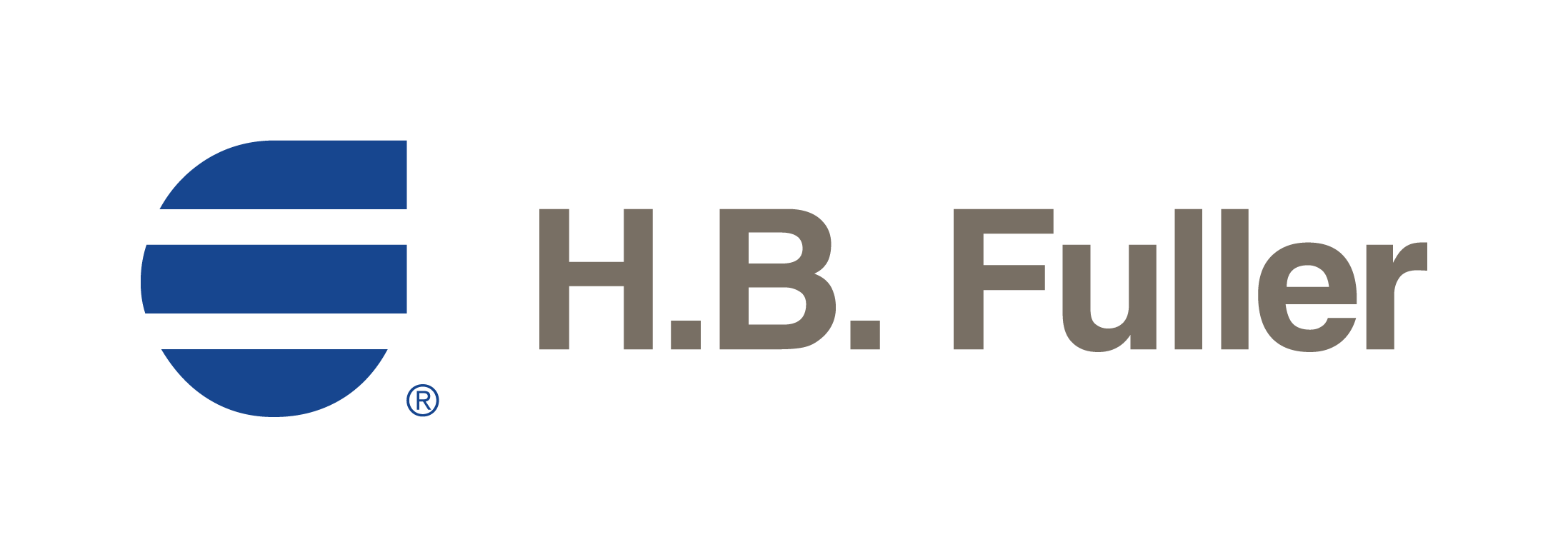
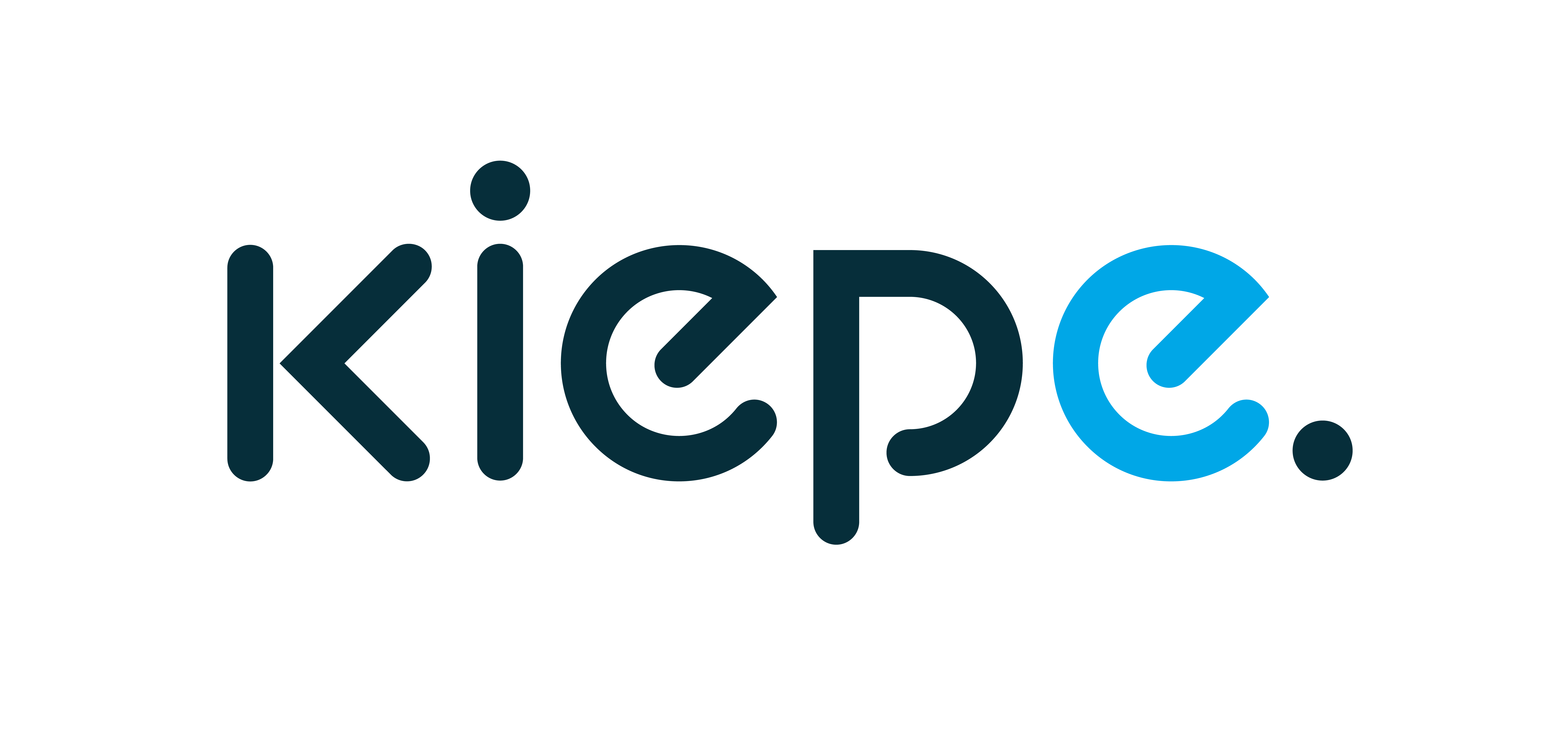
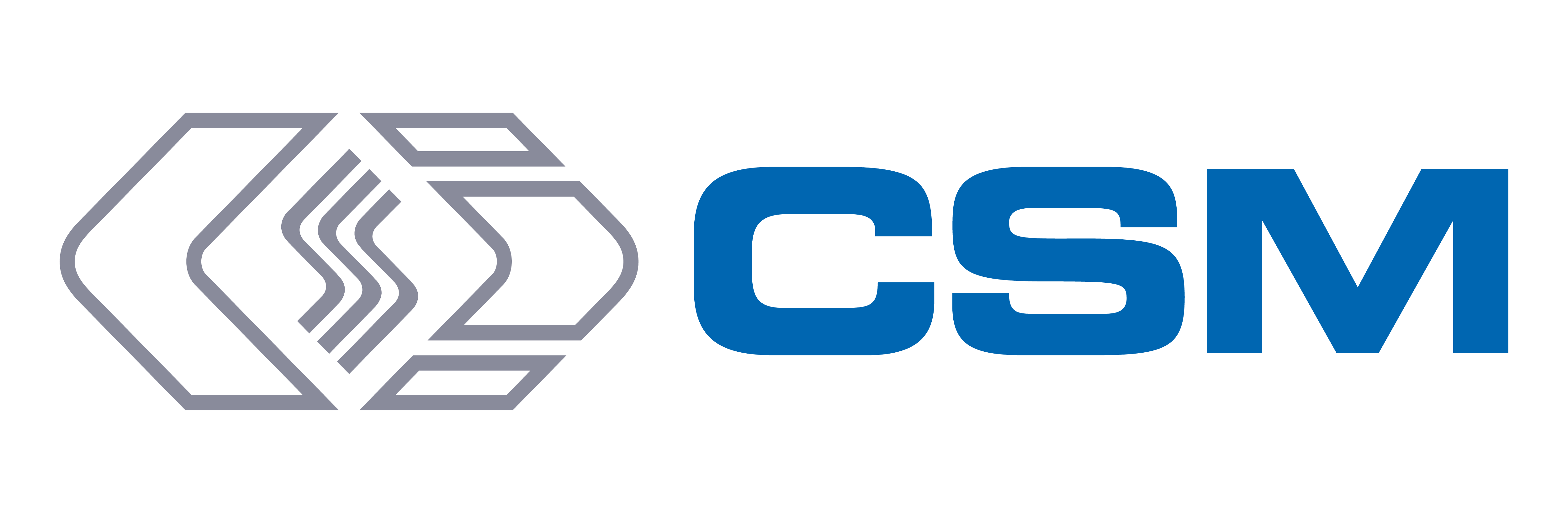